ABSTRACT
The immersed tunnel which is composed of elements has drawn
more attention nowadays because of new advancements and
developments. The elements are prefabricated somewhere else and
floated to the tunnel site to be sunk into the prepared trench.
Each element must line up exactly for the watertight gaskets to
seal properly. The HZM immersed tunnel, a key part of the Hong
Kong-Zhuhai-Macao Bridge (HZMB) that crosses the Pearl River
Estuary and links Hong Kong to the east, and Zhuhai and Macao to
the west, is 6.7 km long, one of the longest immersed tunnel
ever realized in the world. For the construction of such a
long immersed tunnel, particular care should be taken in the
perspective of geodetic control. This paper described the design
and implemention of the geodetic basis and hierarchical surface
control networks. And to decrease the influence of lateral
refraction and to improve the configuration strength and
increase the number of redundant observation compared with
traverse, the design of underground surveying network named
duo-linear joint chain and the results of gyro checks are
presented. In the end HZM immersed tunnel surveying data is
analysed and the performance of the control networks is
demonstrated.
1. INTRODUCTION
When it comes to a large cross-water passage, people always
think of a bridge or a bored tunnel. However, the attention has
now been attracted by a competitive alternative, the immersed
tunnel, because of its rapid advancements and developments (Chen
2002). The immersed tunnel is composed of elements,
prefabricated on shipways, in dry docks, or in improvised
floodable basins, sealed with bulkheads at each end and floated
to the tunnel site to be sunk into the prepared trench and
linked together (Chen 2002, Yanning and Gang 2007). Immersed
tunnels lie only a short distance below water bed level compared
with high bridges or bored tunnels. The overall length of
crossing can therefore be relatively shorter. Immersed
tunnelling involves more operations that can take place
concurrently or overlapping, resulting in a more robust project
planning and more cost effective (Lunniss and Baber 2013).
The Hong Kong-Zhuhai-Macao Bridge (HZMB), crossing the Pearl
River Estuary and linking Hong Kong to the east, and Zhuhai and
Macao to the west, is a combination of bridges, a tunnel and
artificial islands. This crossing consists of three parts, the
offshore bridge and tunnel (the Main Bridge), the three
boundary-crossing facilities (BCFs) in Hong Kong, Zhuhai and
Macao, and the link roads into the three regions. The island and
tunnel section, a key part of the Main Bridge, includes two
artificial islands and an immersed tunnel. This section is
7440.546m in length, of which the immersed tunnel is one of the
most challenging constructions of its kind undertaken in the
world to date.
With a length of approximately 6 km and a depth of about 44 m
(tunnel bottom) below sea level, the immersed tunnel is the
longest and one of the deepest ever realized in the world. The
longitudinal section of the immersed tunnel is shown in Fig. 1.
The standard elements (180×38×11.4 m) for this project are
currently the largest ones (Hu et al. 2015, Zhigang et al.
2016).
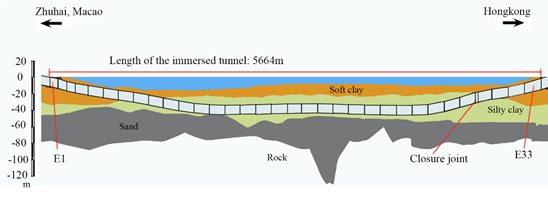
Fig. 1 Longitudinal section of immersed tunnel for the
Hongkong-Zhuhai-Macao Link
The tunnel element is towed to the work-site for installation
and connection to the previous one. The tolerances involved in
the process are tight. Each element must line up exactly for the
watertight gaskets to seal properly, which is performed under
precise control networks, including hierarchical surface control
networks and an underground surveying network. As required, the
relative precision between two adjacent elements is better than
±35mm and the absolute precision of each element position is
better than ±50mm in Hong Kong-Zhuhai-Macao (HZM) immersed
tunnel, regardless of the distance to the portal. And it is
important to note that, unlike other kinds of tunnels, where
alignment at breakthrough is most critical, for immersed tunnel
each element has the same precision requirement. There must be
meticulous geodetic network design, careful field surveying and
rigorous data processing for such high precision requirement.
This paper discusses the geodetic basis and networks design of
HZM immersed tunnel.
This paper is organized as follows. First, the realization of
the geodetic basis, the foundation of all other surveying work,
is described. Next, how hierarchical surface control networks,
the primary global positioning system (GPS) network and the
densified GPS network, were built are presented. Third, the
influence of the lateral reflection is discussed; the
underground surveying network named duo-linear joint chain and
gyro checks are presented. Fourth, the measured data from HZM
immersed tunnel is analyzed and the breakthrough result is
given. Finally, conclusions are described.
2. GEODETIC BASIS
The geodetic basis including a three dimensional reference
system, two independent plane coordinate systems (Bridge and
Tunnel Coordinate System, BCS2010 and TCS2010), a height system,
coordinate transformation parameters connecting to the different
reference frames of Hong Kong, Zhuhai, Macao and the two
independent plane coordinate systems is designed.
HZM link reference system is realized by the ITRF2005, at that
time the most accurate realization of the ITRS. Three
continuously operating reference stations were established
around HZM link in 2010, the purple triangles as shown in Fig.
2. GNSS data were collected after the establishment of the
continuously operating reference stations. Data from seven Hong
Kong & Macao continuously operating reference stations and
nearby stations of the network of the International GNSS Service
(IGS) was included when data processing. IGS stations with
coordinates in the ITRF2005 were used as reference stations.
Therefore, the ITRF2005 was introduced into the project area and
the three dimensional reference system was established for HZM
link.
Then the project ellipsoid was constructed. Based on the
reference system, the reference ellipsoid should approach the
mean elevation plane of the bridge or the tunnel as closely as
possible after ellipsoid transformation. The ellipsoid
parameters are the same as the parameters of WGS-84 ellipsoid.
The geodetic coordinates and 3D rectangular coordinates were
calculated based on the project ellipsoid. The central meridian
of the project was regarded as the central meridian. The
compensation height surfaces of the bridge and tunnel are taken
as the projective plane of BCS2010 and TCS2010 respectively. In
independent reference systems the projection length distortion
can be controlled within ±5mm/km.
The coordinate system currently used is Beijing 1954 in Zhuhai,
HK 1980 in Hong Kong and Macao independent coordinate system in
Macao. The seven-parameter transformation is used to transform
the coordinates between different three-dimensional Cartesian
coordinate systems. The seven parameters were calculated with at
least three points whose coordinates were known before and after
the transformation. The coordinate transformation between
different plane coordinate systems adopts four-parameter
transformation. To obtain the parameters, no less than 2 points
in both systems are needed.
3. SURFACE CONTROL NETWORK
3.1 Primary Control Network
The design of the primary control network, as shown in Figure 2,
consists of 24 GPS stations, including static GPS stations and
continuous reference stations, nine distributed in Zhuhai, four
in Macao and eleven in Hong Kong.
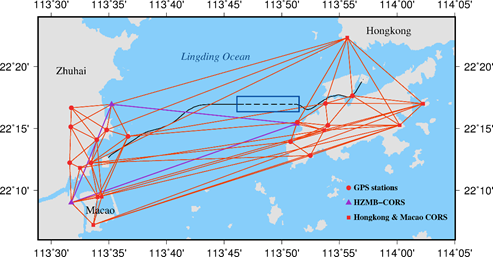
Fig. 2 HZM link primary control network (The blue box is the
area of the immersed tunnel)
The primary control network began to be established in 2008.
During the engineering construction period, the primary control
network is repeat surveyed yearly. It is observed using dual
frequency receiver according to the requirements of Chinese
specifications for global positioning system surveys Class B and
processed using the IGS precise ephemeris. The maximum length of
baselines is more than 40 km. The primary control network is
designed to control the overall HZM link project.
3.2 Densified Control Network
After the primary control network was established the densified
control network as its densification was constructed. Two
artificial islands were designed for the transition between the
bridges and the tunnel (Fig. 3). Each island is approximately
625 m long, less than 200 m at its widest point. The islands
were completed in 2011. The area of the artificial islands is
very complex from a geotechnical point of view. Large land
reclamations in combination with ground improvements are carried
out close to different periods of the project program and a
considerable mutual influence (Hu et al. 2015). Due to the large
reclamations in the area of the islands significant settlement
and displacement can be expected and will develop with time
(Guangqing et al. 2016).
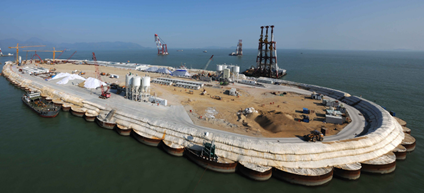
Fig. 3 The west artificial island (taken in Sep. 2012)
An offshore measuring platform was built at about 300 m north of
each artificial island. The difficulty of finding stable
location for stations has resulted in the development of a
strategy for densification of the primary control network.
Control points are established in the artificial islands and
changed as the engineering conditions except two points in each
platform. The points are distributed in such a way that they
provide good positional and azimuthal control convenient to
project implementation. The densified network is measured by
means of GPS, repeating survey every three months and check
survey every month, shortly before the start of the transfer
survey from surface ground into the immersed tunnel, with the
same amount of GPS receivers as stations and adequate duration.
The densified control network when 7th repeating survey is shown
in Fig. 4. It comprises fourteen points deployed in the
artificial islands and measuring platforms. The red triangle
points in platforms are measured together with the primary
control network to obtain their engineering coordinates and are
initial points of the densified network. The distance between
two adjacent points is from several meters to more than seven
kilometers. According to the results of repeating surveys
and check surveys, the relative horizontal positional accuracy
in the network is less than ±2mm at the 99% level of confidence.
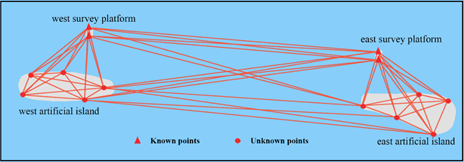
Fig. 4 HZM immersed tunnel densified control network sketch map
4. UNDERGROUND CONTROL NETWORK
4.1 Network design
HZM tunnel immersed part consists of 33 tunnel elements, of
which most have a length of 180 m. The cross-section of the
immersed tunnel is shown in Figure 5, which comprises two
traffic bores and one utility gallery. The typical outer
dimensions are 37.95 m × 11.4 m (Zhigang et al. 2016). There is
good inter-visibility between the two bores because of the
escape doors in every element, as shown in Fig. 6.The immersed
tunnel section measures 5664 m, the cut and cover tunnel
sections on the artificial islands 163 m and open ramps on the
islands 355 m, making the total tunnel length equal some 6.7km.
The tunnel construction began at the west island direction from
the element E1 to E29, then at the east island direction from
the element E33 to E30. The closure joint is between element E29
and E30. The length of west section from the portal to closure
joint is approximately 5.5 km, of east section approximately 1.2
km. In each section an underground network must be built.
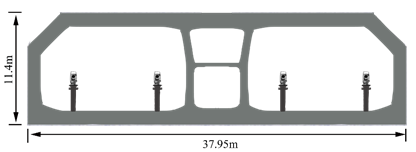
Fig. 5 The cross section of HZM immersed tunnel
The main systematic error source inside the tunnel is the
influence of lateral refraction (Velasco-Gómez et al. 2016). A
considerable gradient of temperature in the direction
perpendicular to the axis of the tunnel may be produced by the
heat transferred from the seawater. The gradient of temperature
depends on many factors, such as the depth of the tunnel below
the sea surface, the type of ventilation system, the thickness
of the tunnel wall and the type of the element. It is hard to
know the exact values of the gradient of temperature in the
immersed tunnels. Theoretically, the gradient of temperature is
believed to be axisymmetric in respect to the axis of the
immersed tunnel. Therefore, as regard to HZM immersed tunnel, to
establish a traverse as close as to the centre line and as far
as possible from the tunnel wall or to run a double traverse on
both sides of the tunnel with the same off-set distance from the
centre line is the easiest way to decrease the influence of
lateral refraction (Chrzanowski 1981; Korittke 1990).
Based on the above principle, simulations are undertaken to
determine the suitable underground control network
configuration. The precisions of angles and distances are
±0.5″and ±1mm+1ppm in the simulations and the side length is
720m, the length of four elements (similarly hereinafter). The
error ellipses of the traverse and double traverse with common
stations are as shown in Fig. 7 (a) and (b). The lateral errors
of the last point of the traverse and double traverse are 51.66
mm and 47.21 mm, beyond the immersed tunnel accuracy
requirements.
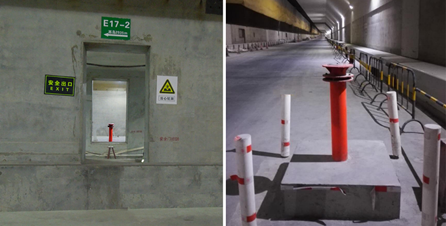
Fig. 6 Inter-visibility between the two bores and steel pillar
with forced centering plate
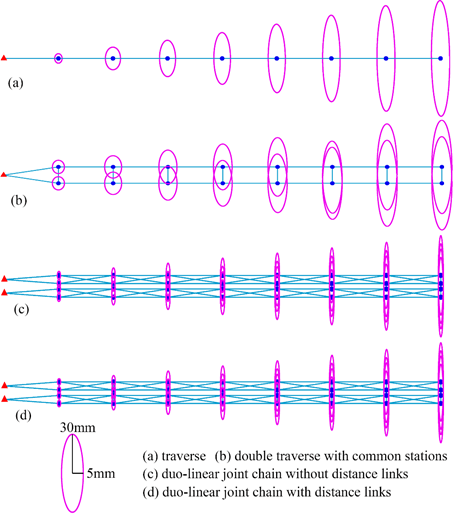
Fig. 7 Error ellipses of underground networks
Based on the double traverse, each traverse is taken place by
the traverse chain to produce a more complicated network,
keeping the entire network axisymmetric in respect to the axis
of the immersed tunnel, for increasing the precision. The new
network is called duo-linear joint chain, as shown in Fig. 7 (c)
and (d). Comparing simulations of the new network without and
with the distance links between the bores are undertaken and
results show that they own almost the same precision of the last
points. The lateral errors are 30.19 mm and 30.17 mm
respectively. The error ellipses are as shown in Fig. 7 (c) and
(d). For the reliability purpose the distance links are
suggested. In this case, the traverse chain in each bore can be
checked by the one in another bore. If it is valid that the
theorem on the symmetrical distribution of the gradients of
temperature, the traverse chains in both bores should be
deflected in the opposite directions. That is to say the overall
adjustment from both traverse chains should be almost free of
refraction error.
A length of 720 m of the west network legs is eventually
selected after severe pre-analyses and mathematical
optimizations of accuracy and a reasonable compromise between
tunnel conditions such as station inter-visibility and accuracy
requirements. A length of 180 m of the east network legs is
selected. Total stations are used for angle and distance
measurements. Steel pillars with forced centering plate are
installed as permanent underground survey control points (Fig.
6). GYROMAT 3000 is used to make gyro checks and to insure the
reliability of results.
4.2 Gyro check
The gyro checks were made in December 2015. Reciprocal
observations with GYROMAT 3000 on same network lines were done.
Angle and distance measurements were done using TS30. The length
of the underground network was 4.3 km and the configuration is
as shown in Fig. 7 (d). The value of the gyrotheodolite constant
was established on the sides of the exterior densified control
network. Table 1 shows the differences between the bearings of
the duo-linear joint chain after an adjustment of the network
with and without gyro observations. The differences between the
bearings increase as the length of network become larger.
However the magnitude is small. The influence of lateral
refraction is decreased effectively by the axisymmetric network.
It is permissible to consider that only random errors exist in
angle and distance observations.
Several years will usually be taken to establish the geodetic
networks for a mega project. For the densified control network
of HZM immersed tunnel, the first measurement was implemented in
November 2012, and the 17th in March 2017. Because significant
displacement and settlement have taken place and there are more
and more buildings in the narrow artificial islands, the GPS
stations in islands have been changed many times so that the
stations were not exactly the same every two measure periods.
Hence, a principle has been put forward that the underground
survey must be implemented instantly after the densified control
network survey, particularly the stations that link the ground
and underground.
5. MEASURED DATA ANALYSIS AND BREAKTHROUGH RESULT
For evaluating the real engineering application effect of the
duo-linear joint chain, the data measured in February 2017
before the closure joint installation is analyzed to estimate
its precision. The length of the underground network was about
5.4 km. The result of constrained network adjustment is

where
is
the estimation of t unknowns, A is the n
by t design matrix with rank t, P is the
weight matrix, l is the constant vector of n
measurements,
is
variance of unit weight,
and
are covariance matrix and cofactor matrix which contain all
accuracy information of the network.
Positional accuracy is taken as the index in this paper. And
the comparison is made with the two double traverses that the
duo-linear joint chain contains. Positional error
is
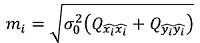
Tab. 1 Gyro checks of duo-linear joint chain
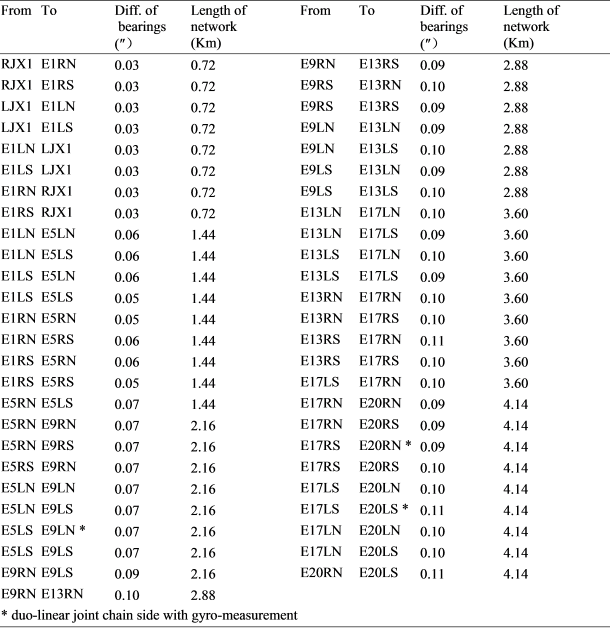
The measured data is processed and analyzed according to
different configurations (the duo-linear joint chain and double
traverse). The results are shown in Fig. 8. Similar to the
simulations, the precision of the duo-linear joint chain is
higher than the precision of double traverse. Suppose ratio is
equal to
,
where
is
the position error of the duo-linear chain point and
is
the position error of the double traverse. The ratio is smaller
than 85% and becomes smaller as the network length increases.
The measured positional error of the last point in the
underground duo-linear joint is 13 mm, much less than the
simulation results shown in Fig. 7. This indicates that the
measured data has higher accuracy than the simulation data. It
should be predicted that the HZM link immersed tunnel alignment
can be controlled well by the duo-linear joint chain.
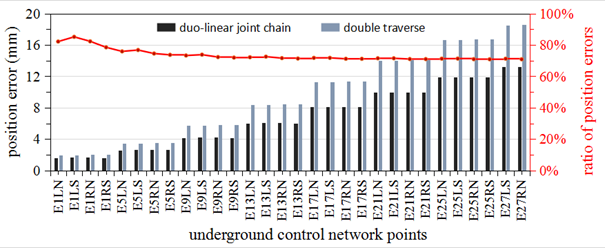
Fig. 8 Results of precision evaluation. ratio=
, where
is the position error of the duo-linear chain point and
is the position error of the double traverse
The closure joint was installed in early May 2017. The
lateral breakthrough error is 12 mm. It is demonstrated that the
design of surface and underground networks is effective.
6. CONCLUSIONS
The installation of elements and alignment control must be
guaranteed by very high precision surface and underground
networks. The stringent accuracy requirements, the rigorous
environment and the scale of construction make HZM immersed
tunnel a very challenging surveying project. Hierarchical
surface control networks design and the duo-linear joint chain
design are discussed in detail in this paper. HZM immersed
tunnel breakthrough surveying data is analyzed and the
performance of the geodetic networks and data processing is
validated well. The conclusions are mainly as follows:
Hierarchical surface control networks, the primary control
network and densified control network, are designed and
constructed. The network observations are done by GPS
techniques. Adequate multiple observations is taken to guarantee
repeatability and reliability.
The underground surveying network, named duo-linear joint
chain, is designed. It has higher precision and reliability with
more redundant observations and stronger configuration than
traverse. This high-level network can be expanded to other
precise engineering projects, including but not limited to other
immersed tunnels in construction and in planning.
The gyrotheodolite usage is the only method to decrease the
influence of refraction for most of tunnels. However, the
gyrotheodolite usage can be omitted according to the result of
the gyro checks in HZM immersed tunnel. The influence of lateral
refraction is decreased effectively by the axisymmetric network.
REFERENCES
Chen, S.Z., 2002. Design and Construction of Immersed Tunnel.
Beijing: China Communications Press.
Chrzanowski, A., 1981. Optimization of the breakthrough accuracy
in tunneling surveys. The Canadian Surveyor, 35(1), pp.5-16.
Guanqing L. and Shengxiang, H., 2016. The breakthrough error
estimation of HongKong-Zhuhai-Macau Bridge immersed tube tunnel.
Science of Surveying & Mapping, 41(12), pp.10-13.
Hu, Z.N., Xie, Y.L. and Wang, J., 2015. Challenges and
strategies involved in designing and constructing a 6km immersed
tunnel: A case study of the Hong Kong–Zhuhai–Macao
Bridge. Tunnelling and Underground Space Technology, 50,
pp.171-177.
Korittke, N. 1990. Influence of Horizontal refraction on
traverse measurements in tunnels with small diameter.
Proceedings of the Second International Workshop on Accelerator
Alignment. Hamburg, Germany, 10–12 September 1990. Hamburg:
Deutsches Elektronen Synchrotron DESY.
Lunniss, R. and Baber, J., 2013. Immersed tunnels. Boca
Raton: CRC Press.
Velasco-Gómez, J., Prieto, J. F., Molina, I., Herrero, T.,
Fábrega, J. and Pérez-Martín, E., 2016. Use of the
gyrotheodolite in underground networks of long high-speed
railway tunnels. Survey Review, 48(350), pp.329-337.
Yanning, W. and Gang, X., 2007. Application and state of the
art of immersed tube tunnels. Modern Tunnelling
Technology, 44(4), pp.1-4.
Zhigang, Z., Wei, L., Hai, J. and Xiaodong, L., 2016. Layout
and Design Techniques of Cross Section for the Large Immersed
Tunnel. Procedia Engineering, 166, pp.37-44.
BIOGRAPHICAL NOTES
Guanqing Li is a Ph.D. candidate of Geodesy
and Surveying Engineering at Wuhan University, China. His
research interests include precise engineering surveying and
deformation monitoring.
Shengxiang Huang is a Professor of School of
Geodesy and Geomatics & Collaborative Innovation Center for
Geospatial Technology at Wuhan University, China. His research
interests include deformation monitoring and disaster
prediction, satellite navigation and positioning technology &
precise engineering surveying.
CONTACTS
Ph.D. candidate Guanqing Li
School of Geodesy and Geomatics, Wuhan University
No. 129, Luoyu Road, Hongshan District
Wuhan, China
Tel. +86 18986140130
Email: liguanqing[at]whu.edu.cn
Prof. Shengxiang Huang
School of Geodesy and Geomatics & Collaborative Innovation
Center for Geospatial Technology, Wuhan University
No. 129, Luoyu Road, Hongshan District
Wuhan, China
Tel. +86 13871202468
Email: sxhuang[at]whu.edu.cn